ABSTRACT
Fine bubble is defined as the small bubbles with a diameter ranging in micro and nanoscale. The sizes of micro bubble are smaller than millimeter and have distinctive properties. The applications of micro bubbles technology has been successfully proved in waste water treatment. More application was investigated in washing process to reduce microorganisms and pesticides. The effectiveness of micro bubble water to reduce E. coli and Salmonella contamination on six Thai fresh vegetables during washing step was conducted. A preliminary study was performed on artificially contaminated coriander, Marsh mint, asparagus, okra, lemongrass and ginger. Washing samples for 15 minutes by micro bubble water at flow rate of 4.5 L/ min successfully reduced both pathogens in these tested vegetables. Second experiment was done to investigate the combination of micro bubbles with sanitizers, sodium hypochlorite, acetic acid and citric acid in washing Chinese kale. The results showed the promising methods to reduce microbial load compared to normal washing, although no significant among the different at concentration levels. These techniques have potential to apply in washing steps to enhance the safety of food, particular in fresh produce process.
Keywords: Micro bubbles, washing produce, foodborne pathogen, sanitizers, produce safety
INTRODUCTION
Microbial contamination in fresh produce
Recently, there has been an increasing consumption of fresh produce worldwide due to the concerning of consumers on their health benefits. Meanwhile food safety problems particularly the pathogenic microorganisms linked to contaminated fresh produce have been repeatedly reported (Kirezieva, et al., 2015). Foodborne pathogens could contaminate on fresh produce at many steps along the production from farm to table at point of consumption. The contamination of foodborne pathogen can arise from environmental, animal or human sources (Gil, et al., 2009), thus, the prevention and control to the contamination on fresh produce has been focused (Fan and Sokorai, 2015).
In terms of food safety not only the significant microbiological contamination issues concerning as threats to health, but also the issues associated with the market of fresh produces, and in particularly international trade. In 2005, 32 out of 244 herbs imported from non-EU countries, sold in London, UK, were contaminated with Salmonella, those including four varieties of basil grown in Thailand. Again in 2006, 5 out of 298 fresh herbs in the UK including coriander, curry leaves, and holy basil that imported from India and Thailand were contaminated with Salmonella (Elviss et al., 2009).
The majority of pathogens that implicated in fresh produce outbreaks are enteric micro-organisms that originated from the gut of warm-blooded animals (Xuetong et al, 2009). For example the enteric pathogens such as Salmonella spp. Among these enteric bacteria, both genus Salmonella and Escherichia coli O157:H7 are the major pathogens contributing to outbreaks of foodborne illness associated with fresh produce (Delbeke et al., 2015; Olaimat and Holley, 2012)
Factors contributing to the contamination in fresh produce
There are numerous factors leading to microbial contamination, human pathogens can be introduced to fresh produce production systems through animal feces, from wildlife or livestock or by the application of manures as fertilizers (Beuchat, 2002; Xuetong et al, 2009). Number of the presence microorganisms differed depending on the type of produce, agronomic practices, geographical area of production, and weather conditions. Other than the hygienic conditions, pre-harvesting, post-harvesting, transportation, further processing and handling of fresh produce can also significantly influence on the microbiota pattern (Ramos et al., 2013).
Leaf topography is an importance factor for microbial adhesion. The surface roughness of the leaves, crack in the cuticle and other damages are often sites at which bacteria colonize. Leaf stomata provide protective niches for the bacteria. E. coli O157:H7 cells have been found that cells could adhere better to cut lettuce leaf surfaces than intact or normal lettuce leaf surfaces (Seo and Frank, 1999). Many reports showed human pathogen can enter stomata and cut edges of fresh produce (Berger et al., 2010; Golberg et al., 2011)as well as the ability of E. coli O157:H7 and Salmonella to internalize or infect the vascular system of growing plant (Olaimat and Holley, 2012).
Washing fresh produce
Fresh produces washing is a significant step for removing soil, debris, and improving the appearance of the commodity. Washing also to lowering the produces’ temperature to limit the development of physiological changes. Moreover, washing helps to reduce the microbial load on the surface which has impact to quality, shelf-life, and safety of the produces (Herdt and Feng, 2009). Washing produces with water or sanitizing agents could help to remove pesticide residues (Sapers, 2014).
In contrast, washing can transfer microbial contaminants to wash water and also to other uncontaminated raw materials, as well as to the processing equipment (Gil et al., 2009).. Adding sanitizing agents to wash water significantly reduce the population of bacterial cells, and thus the risk of cross-contamination (Sapers, 2014). Washing with sanitizers assist to avoid cross-contamination between clean and contaminated product and significant enhance produce hygiene (Gil et al., 2009). Typically a microbial load of fresh produce can be reduced 1 to 2 logs by washing and disinfection. However, washing is restricted to treat the microorganisms on the surface and internalized microorganisms of fresh produces (Lee et al, 2004).
Not all washing methods and washing solutions are effective (Olaimat and Holley, 2012), the success of washing depends on many factors: type of washing, exposure time, the concentration of sanitizing agent, pH, temperature as well the target microorganisms, the characteristics of produce surface, the attachment of cell to produce surface, the formation of resistant biofilms and the internalization of microorganisms (Allende et al., 2008). The challenges of choosing sanitizing treatment are how to reach pathogens on the surface and in subsurface areas of fresh produce in an active formand and at the same time compromise the quality of fresh produce after treatment (Beuchat, 2004).
Sanitizing agents for fresh produces sanitation
Three factors could influence the efficacy of disinfection need to be noted. Firstly, sanitizing agents including pH, temperature, and water quality; secondly the availability of disinfectant in water which are bound to the inorganic and organic compounds; and lastly the accessibility of sanitizing agents to the target microorganisms (Barbeau et al., 2005).
The chosen of chemical sanitizing agent during the washing disinfection step is a critical step for keeping quality and safety of fresh produces. Ideal sanitizing agent should show sufficient level of antimicrobial activity, and be a negligible effect on the sensory quality of the product (Allende et al., 2008). Most of the common type of sanitizers are oxidizers, working by increasing the oxidation potential in the water system, and are considered to be the Generally Recognized As Safe (GRAS).
Chlorine (Cl) is the most widely sanitizing agent that used to sanitize fresh produce (Sapers, G. M., 2014). It is a very potent disinfectant with powerful oxidizing properties. However an antimicrobial activity of chlorine depends on the pH of wash solution, for the best compromise of activity and stability of hypochlorous acid (HOCl) should be potentially control and maintain at the pH of wash water between 6.5 and 7.5. Currently in the fresh produce industry used Sodium hypochlorite (NaOCl) to disinfectant produce at a concentration of 50-200 mgL-1and a contact time of 1-2 min (Beuchat, 1998;Fan and Sokorai, 2015;Goodburn and Wallace, 2013). Another was reported at 50-150 mg/L (Martínez-Hernández et al., 2015). However the chlorination water containing free chlorine between 20 to 200 mg/L in sanitizing solution cannot eliminate pathogens completely, the reduction of 1 to 3 log CFU/g are common (Aruscavage et al., 2006).
Alternative sanitizing agents used for fresh produce such as electrolyzed oxidizing water, chlorine dioxide, ozone, peroxyacetic acid, acidified sodium chlorite or peracetic acid have been studied (Alvaro et al., 2009;Alwi and Ali, 2014;Artés et al., 2009;Gil et al., 2009;Gómez-lópez, 2012;Goodburn and Wallace, 2013;Graça et al., 2011;Hao et al., 2015; Herdt and Feng, 2009;Olaimat and Holley, 2012;Ramos et al., 2013)
Electrolyzed oxidizing water (EO water or EOW) is an emerging decontamination technology. The EOW system was developed in Japan in 1992 and EOW has been attracted much attention in recent years. It is not only effective solution for inactivation of microorganisms but also is considered as environmentally friendly chemical (Cheng et al., 2012). The role in disinfection mechanism of EOW consists of high oxidation-reduction potential (ORP), pH and available chlorine content (ACC) ae well as the OH radical in EOW (Hao et al., 2015;Hao et al., 2012;Stan and Daeschel, 2005;Stan et al., 2005). The acidic EOW which had a low pH (2.5 to 3.5), high ORP (1000 to 1200 mV), high dissolved oxygen and free chlorine (30 to 90 mg/L) has been regarded as an effectively antimicrobial activity (Ding et al ., 2015; Huang et al., 2008).
Chlorine dioxide (ClO2) is a biocide with 2.5 times the oxidation capacity of chlorine (Rodgers et al., 2004). Chlorine dioxide is highly stable and less corrosive than ozone and chlorine (Joshi et al. , 2013). It can apply as an aqueous solution, or gas phase (Sapers, 2014). Moreover, ClO2 is not active to organic compounds (Han et al., 2004; Lee et al. , 2004). FDA approved the formulation of ClO2 as an antimicrobial agent in water (aqueous ClO2) (21CFR173.300) and can use ClO2 for wash fresh fruit and vegetables. However the residual of ClO2 in final produce should not over than 3 mg/L of ClO2 and suggestion to rinse with potable water after treatment with ClO2 (López-Velasco et al., 2012; Sapers, 2014).
Emerging technology: Fine Bubble Technology
Fine bubble is defined as the small bubbles with a diameter ranging in micro and nanoscale. Microbubbles are the small bubbles with diameter between 10 to 50 µm and decreasing in size and lastly disappear under water (Hideki, 2014; Parmar and Majumder, 2013; Takahashi, 2005; Takahashi et al., 2007), while the nanobubbles is evensmaller bubbles with diameter less than 200 nm (Agarwal et al., 2011). Stabilized nanobubbles are created when microbubbles are collapse in aqueous electrolyte solution (Masayoshi, 2014).
Microbubble composts of three main components, gas phase, shell material, and aqueous or liquid phase. The gas phase is referred to the gas inside the bubbles which may be single gas or the combination of gases. The second is the shell material, an aqueous phase surrounding the gas phase. The formation of the bubbles and the mechanical properties of microbubbles depend on the property of shell material. The last important component is aqueous phases which are the liquid or combined solution surrounding the shell material (Parmar and Majumder, 2013). These three components mainly contribute to the properties of microbubbles.
Several interesting characteristics of microbubbles comparing with a millimeter or centimeter-sized bubbles are described. Although the microbubbles are defined as the bubbles having diameter in order of micrometer (µm) (Hideki, 2014; Parmar and Majumder, 2013), however the range of the bubbles diameter can be varies. Usually the microbubbles have a slow rising speed. Besides, the Reynolds number of microbubbles is nearly 1 and its shape is spherical (Hideki, 2014).
Microbubbles could reduce frictional resistance, therfore the coefficient of friction decreased with increased in volume fraction of microbubbles (Hideki, 2014; Parmar and Majumder, 2013). The internal pressure of bubbles depend on the bubbles diameter and surface tension of bubbles. Decreasing of bubbles diameter caused the increasing of internal pressure inside of the bubbles, that may describe by the Young-Lapace equation. The occurrence of this phenomenon can be explained as increasing the gas dissolution will increase the mass transfer rate due to driving force of gas dissolution in the internal pressure (Parmar and Majumder, 2013). Lastly, decreasing microbubbles in water affect an increasing of the large gas-liquid interfacial area and the changes of the physical properties of water (Hideki, 2014).
Microbubbles have been suggested that they possses a negative charged on their surface (Hideki, 2014; Takahashi, 2005). The studied of the zeta potential of microbubbles in aqueous showed the zeta potential of microbubbles in distilled water was -35 mV (Takahashi, 2005). The zeta potential of the particle is a physical property that is possessed by any particle in suspension and emulsion. Usually the zeta potential of emissions used to predict the stability of suspension and emulsion stability. Likewise, the fine bubbles in the solution similar characteristic like the particle in suspension and having a significant negative potential tend to repel the other particle (Parmar and Majumder, 2013).
Similarly to the nanobubbles, they are tiny bubbles with the diameter in nanoscale and smaller size than microbubbles. It is invisible in solution while microbubbles are visible. Before the generation of microbubbles water is clear and its change from the clear solution to cloudy and milky after the generating the microbubbles (Masayoshi, 2014 ; Li, 2007). Then the smaller bubbles are shrunk faster and disappear almost instantaneously, and the nanobubbles are created after the microbubbles collapse. The half-life of nanobubbles depends on the water condition, many researchers are trying to clarify the mechanism of nanobubbles stability but the difficulty is the measurement the properties of these tiny particles (Tsuge, 2014)
There are many fields application research of microbubbles and nanobubbles such as environmental field, industrial field, agricultural field, medical and food industry due to the different function properties. Recently bubble technology is applied in waste water treatment particularly in food industries, and thus this technology influences the quality, production efficiency or cost of food products. In food process the development of foods using microbubble (MB) or micro-nano bubble (MNB) technology is expected to obtain a new characteristic because the quantity and size of the food bubbles may contribute to their appearance, physical properties and texture of the foods. The application extends to control over the growing and pre-postharvest physiological properties of fresh produce, sterilization of food or equipment, besides the treatment of public water and wastewater (Xu et al., 2014).
Newly developed or quality-improved food/food materials are expected to be produced by microstructure control. Regarding to the process of cream production, Kukizaki (2009) described that controlling the surface characteristics of Fine bubbles (Micro- and nano-Bubbles) will contribute to improving the stability of the emulsion. Apart from that fine bubble is expected to be used as a delivery system of functional or aroma components in foods and used as a carrier of drug or antimicrobial agents to maintain food hygiene or safety. Micro bubbles treatments were applied for sterilization, some biocidal gases are often incorporated, therefore with the combined effect of ozone bubbling and ozone nanobubble treatment have been shown significant microbial efficiency. Moreover the combination microbubbles with ozone gas effectively reduced the pesticide residual on fruits and vegetables ( Ikeura et al, 2011a; 2011b and 2013)
The application Micro/Nano bubble on reducing pesticides in fresh produce
In 2011, Ikeura and coworker compared the efficacy of two types of ozone microbubbles generator, the decompression-type and the gas-water circulating-type, to remove the residual pesticide fenitrothion in lettuce, cherry tomatoes and strawberries. The decompression type produces a sufficient amount of gas that dissolved in water under a 3-4 atmospheric pressure to cause a supersaturated condition. Under such a condition, supersaturated gas is unstable and when escapes from the water generating a lot of air bubbles, which are microbubbles. While the gas–water circulation type, gas is introduced into the water vortex, and the formed gas bubbles are broken into microbubbles by breaking the vortex. They found that the concentration of dissolved ozone was higher in the decompression type solution than in the gas–water circulation type solution, although the concentration of dissolved ozone decreased gradually with time. Washing with ozone microbubbles water effectively removed residual fenitrothion in all vegetable samples (Fig. 1), and the decompression type was shown to be better. Hydroxyl radicals that are generated by the collapse of ozone microbubbles in solutions are highly effective at decomposing organic molecules (Ikura et al., 2011a), resulting in pesticides degradation.
Reduction rate of fenitrothion on cherry tomatoes was slightly different from the other vegetables because the dissolved ozone and hydroxyl radicals could hardly penetrate through the thick pericarp of the cherry tomatoes. While strawberries fruit that have a rougher surface and larger surface area than cherry tomatoes, fruits can contact with ozone efficiently, therefore removing fenitrothion are easily compare to tomatoes. The difference in the pesticide-removing effect between the decompression type and the gas–water circulation type may be caused by the difference in the size and the number of the bubbles (Ikura et al., 2011b).
Later Ikura and co-worker (2011b) found ozone microbubbles (OMCB) produced by continuous microbubble generator could retain the concentration of dissolved ozone at 2.0 ppm (Fig 2.), resulting in effectively removal of fenitrothion better than the ozone millibubble (OMLB). Continuous microbubble generator removes the pesticide better than the bath generation or non-continuous generating. Later different type of pesticides were investigated by Ikeura et al. (2013), fenitrothion is represented as organophosphate insecticide while benomyl is carbamate fungicide. Since allowable pesticides residues in food have been implemented in Japan and other countries, including persimmon wrapping leaves for special sushi in Nara, Japan. Red and green persimmon leaves were chosen as a sample model.
The pesticide-spiked persimmon leaves were subjected to the treatments by different micro bubble generation methods. Ozone concentrations in water varied from 0.2, 0.5, 1.0 and 2 ppm, at immerge times 5, 10 or 15 min, were subjected to leaf samples. The residual of fenitrothion on persimmon leaves were decreased when increased washing time from 5 to 15 min, and again continuous generating the microbubbles was shown better effective removal of the fenitrothion than batch generating, likewise the reduction of benomyl on these leaves. No effect of bubbling or continuous microbubbles during treatment to the color and the pulling strength of Persimmon leaves were found (Ikura et al., 2011b).
Experiment I : Removal of foodborne pathogen in Vegetables using combination of sanitizers and microbubbles. (Mahakarnchanakul et al., 2010)
Six types of popular Thai fresh produce were selected and classified into three types; leafy type: coriander (Coraindrum sativum) and peppermint or Marsh mint or Thai mint (Mentha arvensis); Pod and stem type: asparagus (Asparagus officinalis) and okra (Abelmochus esculentus); Root type: ginger (Zingiber officinale) and lemongrass (Cymbopogon citrates).
All fresh produce were stored at 5°C and placed on the laboratory bench for 30 min, then selected similar size and shape. The 18 h suspension of Salmonella Hvittingfoss and Escherichia coli were prepared for inoculum, the final load on each vegetable type was expected to be 103-104 CFU/ml. No wash before inoculating. Then, vegetables were inoculated by adding culture suspension in Polyethylene bag, manual shaking for 2 min, placing under a laminate flow cabinet to let them dry for 30 min. Samples were analyzed for initial loads.
Micro bubble water was prepared by circulating the tap water through a generator in sterile plastic tank (5L). MB generator was obtained from Shigen Kaihatsu Co., Ltd., Japan. Five hundred grams of each sample was submerged in 5 L of micro bubble water (at flow rate of 4.5 L/min) by ratio 1:10 and, agitating for 15 min. Then vegetables were drained and dried under laminate flow cabinet at 20± 3oC (65-70% relative humidity) for 30 min before microbial analyze.
Total viable counts were determined on Plate Count Agar (PCA) while E. coli was counted on McConkey agar, likewise S. Hvittingfoss on Xylose lysine desoxycholate (XLD). Plates were incubated at 35 °C for 24 h before enumeration the survivors (Figure 4). All samples were enumerated by rinse test to avoid the natural antimicrobial from smashed vegetables.
The fragile leaves of coriander and Marsh mint are easily damaged from the harsh washing process. Washing by using MB water showed distinctively good appearance and fresh green leaves due to dissolved oxygen during bubbles generating. The reduction of microbial population by washing with micro bubble water was better than normal washing with tap water. MB treatments could reduce microflora on coriander by 87.6% log reduction, while tap water could reduce microflora load about 56% log reduction. While washing Marsh mint showed microbial reduction by tap water and micro bubbles treatment as 95 and 80 % reduction, respectively (Figure 3A and 3B).
Thai asparagus and okra were known as major exporting produce particularly for Japan market. Washing with MB water and tap water could reduce natural microflora on asparagus and okra. The reductions of total microbial on asparagus and okra using MB water were 99.6 and 98 % compared to washing with tap water, which gave reduction less by 92 and 95 %. (Figure 3C, and 3D). The MB showed the high efficiency in reducing normal flora in asparagus and okra by 1.7-2.4 log CFU/g.
In case of root type, lemongrass and ginger, normally the natural load was high as 6-7 log CFU/ml due to the nature of root plants. Rough and cracked surfaces of ginger prone to be heavy contaminated with soil and difficult to remove soil, resulting in high microbial population and E. coli presence. Fig 3E and 3F showed the remarkably reduction of microflora on lemongrass and ginger samples. Before washing with MB, microflora on lemongrass and ginger were 5.8 and 6.8 log CFU/ml and after washing population was reduced to 4.3 and 5.8 log CFU/ml (97 and 89% reduction). Washing lemongrass and ginger using tap water showed slightly less reduction by 93 to 94%.
In conclusion the number of total bacterial count (microflora) deposited on surface of tested vegetables was contaminated from 105 to 107 CFU/ml, microbial population was varies depending on the different physiology of plant. Each type of vegetables has different in texture and level of microbial contamination, besides the presence of small fur on Marsh mint leaves, or the crevices of surfaces of ginger resulting in the possible habitat. The chance of soil contact during harvesting may also cause the high microbial contamination in produce. The efficiency of MB washing varied from 78 to 99.6% and presented better microbial reduction comparing to tap water wash at 56 to 95.6%, respectively.
Artificially inoculation acquired the initial load of Escherichia coli (E. coli) and Salmonella on coriander and Marsh mint by 2.5 to 3.2 and 4.2 to 4.6 log CFU/ml. The reductions of E. coli and Salmonella on coriander after MB washing are shown in Figure 4A and 4B. Populations of E. coli on coriander decreased more than 90.0% or 1 log reduction after washing with MNB water, as well as tap water. Salmonella on coriander washed with MNB water and tap water was decreased by 99.5% and 99.0%, respectively.
The effect of MB water and tap water for reducing Marsh mint contaminated with E. coli and Salmonella were shown in Figure 4C and 4D. E. coli was reduced by 95.3 % reductions after MB washing, while tap water was by 73%. Results showed better Salmonella reduction by 98 to 99.8 % with MB water and tap water. Different level reduction between these two strains may result from their ability to attach on produce surface which may affect to less washing out, particularly E. coli.
The best reduction among the six types of tested vegetables was found in asparagus inoculated with E. coli. Completely reduction from the initial of 2.2-2.7 resulting in 100 % reduction (not detect at dilution of 10-1) after washing with MB and tap water (Fig 5A). However the effect of washing might not be great compared to Salmonella. Washing with MB water and tap water assisted to reduce Salmonella populations on asparagus by 74 and 80 % (Figure 5B). Likewise less reduction when washing okra with MB and tap water, microbial reduction was 52 to 86%. Great result was shown when using MB as washing water, 100% Salmonella reduction on okra while using tap water gave 98 %. (Fig 5C and 5D)
The initial population of E. coli and Salmonella on Lemongrass were 2.4 to 2.5 log CFU/ml and 3.3 to 3.7 log CFU/ml (Fig 6A and 6B). Reduction of Salmonella was 98 % reduction. MB water washing could be the promising method to reduce E. coli and Salmonella on lemongrass, as well as 98 % reduction on E. coli and 90% Salmonella on ginger (Fig 6C and 6D).
The advantage of using MNB water as washing agent is the possible on less chemical agent added. This preliminary study was conducted to investigate the possibility and feasibility of Micro bubble water washing to replace chemical in order to sanitize fresh produce. The results revealed that MB water contribute to the promising washing agents on microbial reduction which may be potentially applied to the industry and food services.
However, it was found that additional factors such as water flow rate and washing time assisted to reduce the microbial load. Application in industry need to consider all factors involved in washing produce. Apart from the chemical use, the success of the washing depends on several factors such as target microorganisms, characteristics of produce surface, attachment of cells to produce surface, formation of resistant biofilms and internalization of microorganisms (Allende et al., 2008).
Expeiment II: Effect of combined microbubbles and various sanitizer agents to reduce Escherichia coli on Chinese kale (Klintham et al., 2013)
E. coli TISTR780 and Chinese kale was chosen as the model in this study. The final load on Chinese kale was 5-6 log CFU/mg. The ratio of 200g of Chinese kale per 400 ml of culture suspension was mixed in PE plastic bag and then let it soaked for 15 min, before let them dry in laminar flow cabinet for 20 min. Chinese kale samples were MB treated and the samples were taken for E. coli analysis. Washing condition was done as; 100g of Chinese kale to wash water 10000 mL, with washing time 5 min under continuous generated the microbubbles.
The combination treatments between generated microbubbles and various concentration of NaOCl (50 ppm and 100 ppm), Acetic acid and Citric acid (0.5% and 1.0% w/v) in washing contaminated kale showed remarkably the efficiency of sanitizer to eliminate E. coli on Chinese kale compared to normal washing by tap water. Using the microbubbles water without or with chemical added have no significantly affect microbial reduction, especially E. coli from Chinese kale, however, increasing the concentration of sanitizers (NaOCl, Acetic acid and Citric acid) in washing process contributed to be better microbial reduction (Fig. 7).
Recent experiment: Determination on the efficacy of fine bubbles combined with sanitizers on reducing Salmonella Typhimurium and Escherichia coli on Thai Mint and Sweet Basil
Recent study by Klintham et al. 2014 (unpublished) was performed to determine the effect of washing by using the fine bubbles combined with two types of sanitizers on reducing Salmonella Typhimurium and Escherichia coli on Thai Mint and Sweet Basil. Acidic electrolyte water (20 and 40 ppm free chlorine, AEO), and chlorine dioxide (3 and 5 ppm ClO2) combined with batch bubble generated by IDEC (Model UFBGALF™) were investigated. The condition of washing was at 5 min with shaking (60 rpm at 25±2°C).
Determination of oxidation-reduction potential (ORP) in AEO at 20 and 40 ppm AEO were 1090-1200 mV (pH 2.9-3.0) and 1110-1260 mV (pH 2.7-2.9), respectively, which presented active oxidation, while chlorine dioxide has less ORP value, as 750-850 mV at 3 ppm (pH 5.8), and 810-890 mV at 5 ppm (pH 5.1). After washing ORP value were decreased although the pH of wash water were slightly increased. Using 40 ppm AEO with fine bubble treatment effectively reduced Salmonellae cells on sweet basil and Thai mint with 90.7% and 99.5% log reduction respectively (P≤0.05). Compared to the results on microbial reduction by aqueous ClO2, when combined with fine bubbles, washing treatments could reduce Salmonellae on sweet basil and Thai mint with 99.0% and 90.3% log reduction respectively. Noticeably, E. coli washing with AEO was reduced to less than 74 % and 86 %, similar to the effect of 5 ppm ClO2 on E. coli reduction (9% and 16%). None of microbes was observed in wash water, which showed that adding these two sanitizers in bubble water resulting in strong antimicrobial effects on planktonic bacteria. Although tap water with and without FB also showed microbial reduction, but there are still viable cells 5-6 log10 CFU/ml found in wash water .
Conclusions
Fine bubble technology provides the opportunities to apply in agriculture and food products. Using of the fine bubbles technology has unclear mechanism on pesticides degradation, but Japanese laboratory showed remarkably pesticides reduction when combining fine bubbles with oxidizing agents like ozone. Recently Thai research group applied combination of fine bubbles with chemicals like chlorine compounds, organic acids, and others oxidizing agents. Results showed this technology assist to reduce the pathogenic microorganisms in fresh vegetables. More studies need to be done to further clarify the antimicrobial mechanism, and to control possible factors to maximize the utilization of this technology. This technology has the potential to decrease chemical use, and may be considered as the friendly environmental technology. Lastly, the challenge of the fine bubbles technology is to find appropriate use, and to apply to other food process.
References
Agarwal, A., W.J. Ng and Y. Liu, 2011. Principle and applications of microbubble and nanobubble technology for water treatment. Chemosphere 84(9): 1175-1180.
Allende, A., M.V. Selma, F. López-Gálvez, R. Villaescusa and M.I. Gil, 2008. Role of commercial sanitizers and washing systems on epiphytic microorganisms and sensory quality of fresh-cut escarole and lettuce. Postharvest Biology and Technology 49(1): 155-163.
Alvaro, J.E., S. Moreno, F. Dianez, M. Santos, G. Carrasco and M. Urrestarazu, 2009. Effects of peracetic Acid disinfectant on the postharvest of some fresh vegetables. Journal of Food Engineering 95(1): 11-15.
Alwi, N.A. and A. Ali, 2014. Reduction of Escherichia coli O157, Listeria monocytogenes and Salmonella enterica Sv. Typhimurium populations on fresh-cut bell pepper using gaseous ozone. Food Control 46(0): 304-311.
Aruscavage, D., K. Lee, S. Miller and J.T. LeJeune, 2006. Interactions affecting the proliferation and control of human pathogens on edible plants. Journal of Food Science. 71(8): R89-R99.
Barbeau, B.,R. Desjardins, C. Mysore and M. Prévost, 2005. Impacts of water quality on chlorine and chlorine Dioxide efficacy in natural waters. Water Research 39(10): 2024-2033.
Berger, C.N., S.V. Sodha, R.K. Shaw, P.M. Griffin, D. Pink, P. Hand and G. Frankel, 2010. Fresh fruit and vegetables as vehicles for the transmission of human pathogens. Environ Microbiol 12(9): 2385-2397.
Beuchat, L.R.,1998. Surface decontamination of fruits and vegetables eaten raw: A review. Food safety unit, World Health Organization.
Beuchat, L.R. 2002, Ecological factors influencing survival and growth of human pathogens on raw fruits and vegetables. Microbes and Infection 4(4): 413-423.
Cheng, K.C., S.R.S. Dev, K.L. Bialka and A. Demirci, 2012. Chapter 19-Electrolyzed oxidizing water for microbial decontamination of food pp. 563-591, in A. Demirci and M. O. Ngadi, eds. Microbial Decontamination in the Food Industry. Woodhead Publishing.
Delbeke, S., S. Ceuppens, L. Jacxsens and M. Uyttendaele, 2015. Microbiological analysis of pre-packed sweet basil (Ocimum basilicum) and Coriander (Coriandrum sativum) leaves for the presence of Salmonella spp. and shiga toxin-producing E. coli. International Journal of Food Microbiology 208(0): 11-18.
Ding, T., Z. Ge, J. Shi, Y.-T. Xu, C.L. Jones and D.-H. Liu, 2015. Impact of slightly acidic electrolyzed water (Saew) and ultrasound on microbial loads and quality of fresh fruits. LWT - Food Science and Technology 60(2, Part 2): 1195-1199.
Elviss, N.C., C.L. Little, L. Hucklesby, S. Sagoo, S. Surman-Lee, E. de Pinna and E.J. Threlfall, 2009. Microbiological study of fresh herbs from retail premises uncovers an international outbreak of Salmonellosis. International Journal of Food Microbiology 134(1-2): 83-88.
Fan, X. and K. J. Sokorai, 2015. Formation of trichloromethane in chlorinated water and fresh-cut produce and as a result of reaction with citric acid. Postharvest Biology and Technology 109(0): 65-72.
Gil, M.I., M.V. Selma, F. Lopez-Galvez and A. Allende, 2009. Fresh-cut product sanitation and wash water disinfection: Problems and solutions. Int J Food Microbiol 134(1-2): 37-45.
Golberg, D., Y. Kroupitski, E. Belausov, R. Pinto and S. Sela, 2011. Salmonella Typhimurium internalization is variable in leafy vegetables and fresh herbs. Int J Food Microbiol 145(1): 250-257.
Gómez-López, V.M., M.I. Gil, L. Pupunat and A. Allende, 2015. Cross-contamination of Escherichia coli O157:H7 is inhibited by electrolyzed water combined with salt under dynamic conditions of increasing organic matter. Food Microbiology 46(0): 471-478.
Goodburn, C. and C.A. Wallace, 2013. The microbiological efficacy of decontamination methodologies for fresh produce: A review. Food Control 32(2): 418-427.
Graça, A., M. Abadias, M. Salazar and C. Nunes, 2011. The use of electrolyzed water as a disinfectant for minimally processed apples. Postharvest Biology and Technology 61(2-3): 172-177.
Han, Y., T.L. Selby, K.K. Schultze, P.E. Nelson and R.H. Linton, 2004. Decontamination of strawberries using batch and continuous chlorine dioxide gas treatments. Journal of Food Protection 67(11): 2450-2455.
Hao, J., S. Qiu, H. Li, T. Chen, H. Liu and L. Li, 2012. Roles of hydroxyl radicals in electrolyzed oxidizing water (EOW) for the inactivation of Escherichia coli. International Journal of Food Microbiology 155(3): 99-104.
Hideki, T., 2014. Characteristics of microbubbles pp. 3-10, in. H. Tsuge (ed.) Micro- and Nanobubbles:Fundamental and application. Pan Stanford Publishing Pte. Ltd.
Huang, Y.-R., Y.-C. Hung, S.-Y. Hsu, Y.-W. Huang and D.-F. Hwang, 2008. Application of electrolyzed water in the food Industry. Food Control 19(4): 329-345.
Ikeura, H., F. Kobayashi and M. Tamaki, 2011a. Removal of residual pesticides in vegetables using ozone microbubbles. J Hazard Matter 186(1): 956-959.
Ikeura, H., F. Kobayashi and M. Tamaki, 2011b. Removal of residual pesticide, Fenitrothion, in vegetables by using ozone microbubbles generated by different methods. Journal of Food Engineering 103(3): 345-349.
Ikeura, H., S. Hamasaki and M. Tamaki, 2013. Effects of ozone microbubble treatment on removal of residual pesticides and quality of Persimmon leaves. Food Chem 138(1): 366-371.
Joshi, K., R. Mahendran, K. Alagusundaram, T. Norton and B.K. Tiwari, 2013. Novel disinfectants for fresh produce. Trends in Food Science & Technology 34(1): 54-61.
Herdt, J. and H. Feng, 2009. Aqueous antimicrobial treatments to improve fresh and fresh - cut produce safety pp. 433 in Xuetong F., B. A. Niemira, C. J. Doona, F. E. Feeherry and R. B. Gravani (eds). Microbial Safety of Fresh Produce. Blackwell Publishing and the Institute of Food Technologists. USA.
Kirezieva, K., P.A. Luning, L. Jacxsens, A. Allende, G.S. Johannessen, E.C. Tondo, A. Rajkovic, M. Uyttendaele and M.A.J.S. van Boekel, 2015. Factors affecting the status of food safety management systems in the global fresh produce chain. Food Control 52(0): 85-97.
Lee, S.-Y., M. Costello and D.-H. Kang, 2004. Efficacy of chlorine dioxide gas as a sanitizer of lettuce leaves. Journal of Food Protection 67(7): 1371-1376.
López-Velasco, G., A. Tomás-Callejas, A. Sbodio, F. Artés-Hernández and T.V. Suslow, 2012. Chlorine dioxide dose, water quality and temperature affect the oxidative status of tomato processing water and its ability to inactivate Salmonella. Food Control 26(1): 28-35.
Martínez-Hernández, G.B., J. Navarro-Rico, P.A. Gómez, M. Otón, F. Artés and F. Artés-Hernández, 2015. Combined sustainable sanitising treatments to reduce Escherichia coli and Salmonella Enteritidis growth on fresh-cut Kailan-hybrid Broccoli. Food Control 47(0): 312-317.
Mahakarnchanakul, W., P. Klintham1, S. Tongchitpakdee and W. Chinsirikul, 2010. Micro-/Nano- Bubble Technology: An Alternative for sanitizing fresh fruits and vegetables and other potential applications in Thailand. Chapter 1. Feasibility Study on Using Micro-/Nano- Bubble Water to Eliminate Foodborne Pathogen on Fresh Produce. Research project under National Metal and Materials Technology Center (MTEC) National Science and Technology Development Agency (NSTDA) and NEDO. NEDO report. Thailand.
Klintham, P., W. Mahakarnchanakul, S. Tongchitpakdee and W. Chinsirikul, 2013. Application of microbubbles in washing process fro microbial reduction on Thai fresh vegetables pp. 56. in Proceeding of the International Conference on Postharvest Technology, Food chemistry and Processing.: Developing the Supply Chain towards More Healthy Food. 11-13 Nov 2013, at Hanoi University of Agriculture, Hanoi, Vietnam.
Masayoshi, T. 2014. Nanobubbles: An Introduction pp. 307-315, in. H. Tsuge (ed.) Micro- and Nanobubbles:Fundamental and application. Pan Stanford Publishing Pte. Ltd.
Olaimat, A.N. and R.A. Holley, 2012. Factors influencing the microbial safety of fresh produce: A review. Food Microbiol 32(1): 1-19.
Parmar, R. and S.K. Majumder, 2013. Microbubble generation and microbubble-aided transport process intensification-a state-of-the-art report. Chemical Engineering and Processing: Process Intensification 64(79-97).
Ramos, B., F.A. Miller, T.R.S. Brandão, P. Teixeira and C.L.M. Silva, 2013. Fresh fruits and vegetables-an overview on applied methodologies to improve its quality and safety. Innovative Food Science & Emerging Technologies 20(0): 1-15.
Rodgers, S.L., J.N. Cash, M. Siddiq and E.T. Ryser, 2004. A comparison of different chemical sanitizers for inactivating Escherichia coli O157:H7 and Listeria monocytogenes in solution and on apples, lettuce, strawberries, and cantaloupe. Journal of Food Protection 67(4): 721-731.
Sapers, G.M., 2014. Chapter 17 - Disinfection of contaminated produce with conventional washing and sanitizing technology pp. 389-431, in K. R. Matthews, G. M. Sapers and C. P. Gerba (eds). The Produce Contamination Problem. Academic Press, San Diego, USA
Seo, K.H. and J.F. Frank, 1999. Attachment of Escherichia coli O157:H7 to lettuce leaf surface and bacterial viability in response to chlorine treatment as demonstrated by using confocal scanning laser microscopy. Journal of Food Protection 62(1): 3-9.
Takahashi, M., 2005. Ζ Potential of microbubbles in aqueous solutions: Electrical properties of the gas−water interface. The Journal of Physical Chemistry B. 109(46): 21858-21864.
Takahashi, M., K.Chiba and P. Li, 2007. Free-radical generation from collapsing microbubbles in the absence of a dynamic stimulus. The Journal of Physical Chemistry B. 111(6): 1343-1347.
Takahashi, M.,2014. Chapter 11 Nanobubbles:An introduction pp.307-317, in. H. Tsuge (ed.) Micro- and Nanobubbles:Fundamental and application. Pan Stanford Publishing Pte. Ltd.
Tsuge, H.,2014. Chapter 2 Formation and analysis ofmicrobubbles pp.11-83, in. H. Tsuge (ed.) Micro- and Nanobubbles:Fundamental and application. Pan Stanford Publishing Pte. Ltd.
Xu, Q., N. Nobutaka and T. Shiina, 2014. Chapter 7 Food Industry pp.207-217, in. H. Tsuge (ed.) Micro- and Nanobubbles:Fundamental and application. Pan Stanford Publishing Pte. Ltd.
Xuetong, F., B.A.N., C.J. Doona, F.E. Feeherry and R. B. Gravani, 2009. Microbial Safety of Fresh Produce, pp. 445. in J. H. Feng, eds. Aqueous Antimicrobial Treatment to Improve Fresh and Fresh-Cut Produce Safety. Blackwell Publishing and Institute of Food Technologist. USA.
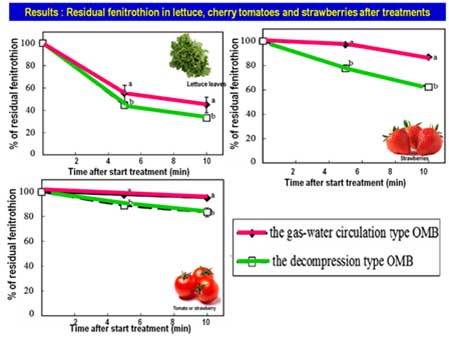
Fig. 1. The residual percentage of fenitrothion (FT) in lettuce (A), cherry tomatoes (B) and strawberries (C) at 5 and 10 min after the immersion into the solutions of ozone micro bubble (OMB) treatments by using the gas–water circulation type and the decompression type. Modified from: Ikeura et al., 2011a.
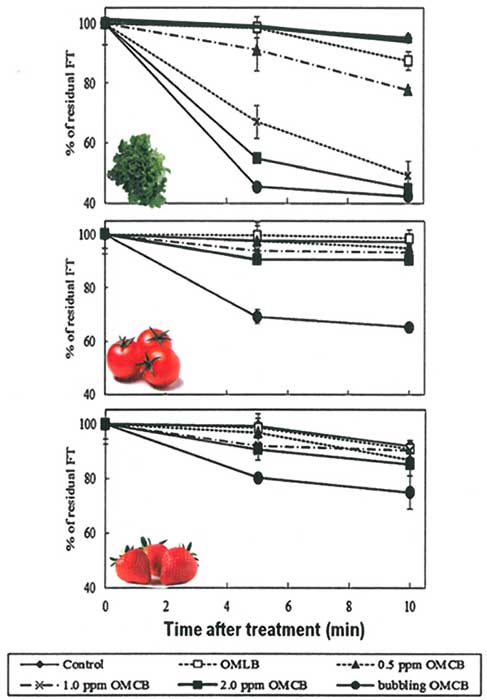
Fig. 2. The residual fenithothion (FT) on lettuce, cherry tomatoes and strawberry treated with the Ozone microbubbles (OMCB) and Ozone millibubbles (OMLB) solutions. Modified from: Ikeura et al., 2011b.
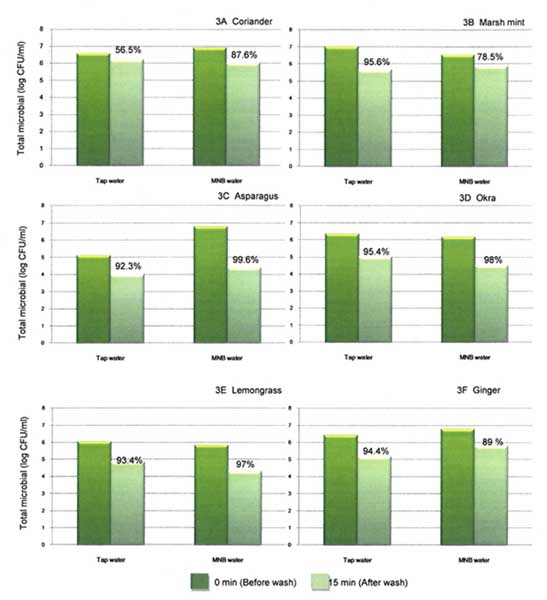
Fig. 3. Populations of total microbial count on un-inoculum coriander (3A), Marsh mint (3B), asparagus (3C), okra (3D), lemongrass (3E), and ginger (3F) before (0 min) and after (15 min) washing by tap water and MNB water.
Ref: Mahakarnchanakul et al., 2010.
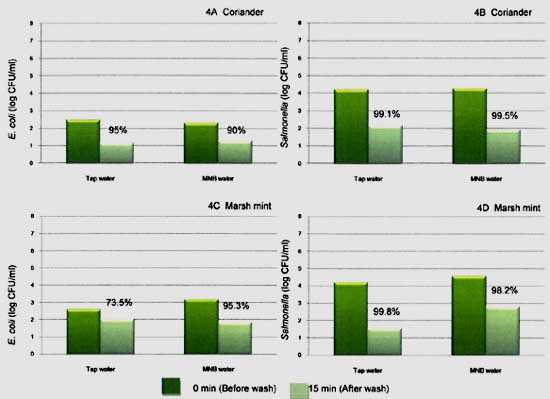
Fig. 4. Populations of E. coli on coriander (4A), Marsh mint (4C), and population of Salmonella on coriander (4B), Marsh mint (4D) before (0 min) and after (15 min) washing by tap water and MNB water.
Ref: Mahakarnchanakul et al., 2010.
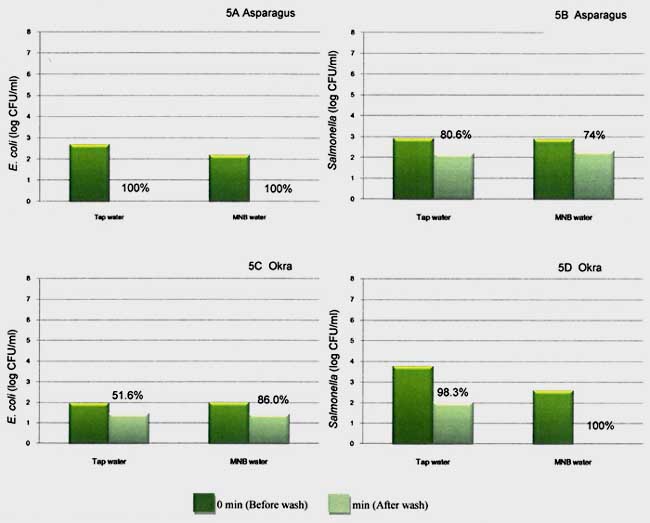
Fig. 5. Populations of E. coli on asparagus (5A), okra (5C), and population of Salmonella on asparagus (5B), okra (5D) before (0 min) and after (15 min) washing by tap water and MNB water.
Ref: Mahakarnchanakul et al., 2010
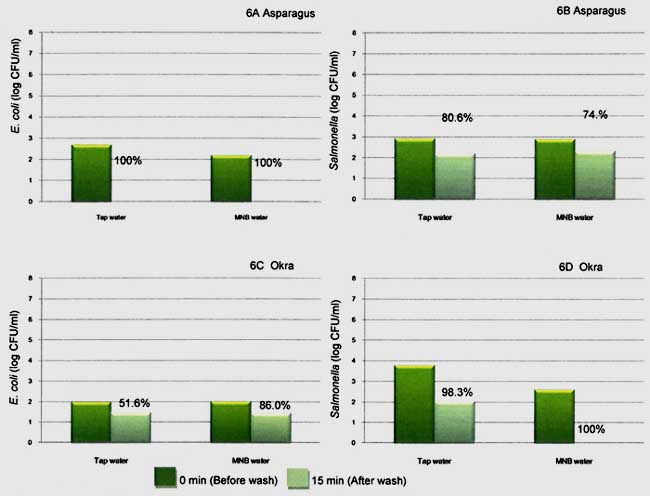
Fig. 6. Populations of E. coli on asparagus (6A), okra (6C), and population of Salmonella on asparagus (6B), okra (6D) before (0 min) and after (15 min) washing by tap water and MNB water.
Ref: Mahakarnchanakul et al., 2010.
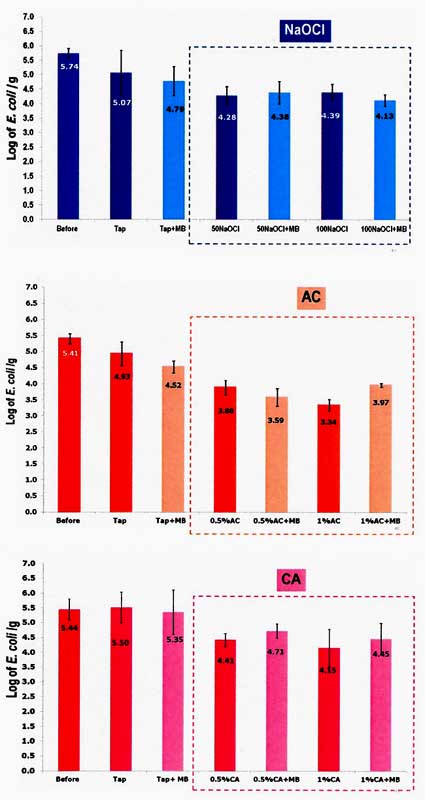
Fig. 7. Survivor of E.coli on Chinese kale after treatments.
Ref: Klintham et al., 2013.
Submitted for the FFTC-KU International Workshop on Risk Management on Agrochemicals through Novel Technologies for Food Safety in Asia, November 10-14, Sampran Riverside, Nakorn Pathom, Thailand |
Using Sanitizer and Fine bubble Technologies to Enhance Food Safety
ABSTRACT
Fine bubble is defined as the small bubbles with a diameter ranging in micro and nanoscale. The sizes of micro bubble are smaller than millimeter and have distinctive properties. The applications of micro bubbles technology has been successfully proved in waste water treatment. More application was investigated in washing process to reduce microorganisms and pesticides. The effectiveness of micro bubble water to reduce E. coli and Salmonella contamination on six Thai fresh vegetables during washing step was conducted. A preliminary study was performed on artificially contaminated coriander, Marsh mint, asparagus, okra, lemongrass and ginger. Washing samples for 15 minutes by micro bubble water at flow rate of 4.5 L/ min successfully reduced both pathogens in these tested vegetables. Second experiment was done to investigate the combination of micro bubbles with sanitizers, sodium hypochlorite, acetic acid and citric acid in washing Chinese kale. The results showed the promising methods to reduce microbial load compared to normal washing, although no significant among the different at concentration levels. These techniques have potential to apply in washing steps to enhance the safety of food, particular in fresh produce process.
Keywords: Micro bubbles, washing produce, foodborne pathogen, sanitizers, produce safety
INTRODUCTION
Microbial contamination in fresh produce
Recently, there has been an increasing consumption of fresh produce worldwide due to the concerning of consumers on their health benefits. Meanwhile food safety problems particularly the pathogenic microorganisms linked to contaminated fresh produce have been repeatedly reported (Kirezieva, et al., 2015). Foodborne pathogens could contaminate on fresh produce at many steps along the production from farm to table at point of consumption. The contamination of foodborne pathogen can arise from environmental, animal or human sources (Gil, et al., 2009), thus, the prevention and control to the contamination on fresh produce has been focused (Fan and Sokorai, 2015).
In terms of food safety not only the significant microbiological contamination issues concerning as threats to health, but also the issues associated with the market of fresh produces, and in particularly international trade. In 2005, 32 out of 244 herbs imported from non-EU countries, sold in London, UK, were contaminated with Salmonella, those including four varieties of basil grown in Thailand. Again in 2006, 5 out of 298 fresh herbs in the UK including coriander, curry leaves, and holy basil that imported from India and Thailand were contaminated with Salmonella (Elviss et al., 2009).
The majority of pathogens that implicated in fresh produce outbreaks are enteric micro-organisms that originated from the gut of warm-blooded animals (Xuetong et al, 2009). For example the enteric pathogens such as Salmonella spp. Among these enteric bacteria, both genus Salmonella and Escherichia coli O157:H7 are the major pathogens contributing to outbreaks of foodborne illness associated with fresh produce (Delbeke et al., 2015; Olaimat and Holley, 2012)
Factors contributing to the contamination in fresh produce
There are numerous factors leading to microbial contamination, human pathogens can be introduced to fresh produce production systems through animal feces, from wildlife or livestock or by the application of manures as fertilizers (Beuchat, 2002; Xuetong et al, 2009). Number of the presence microorganisms differed depending on the type of produce, agronomic practices, geographical area of production, and weather conditions. Other than the hygienic conditions, pre-harvesting, post-harvesting, transportation, further processing and handling of fresh produce can also significantly influence on the microbiota pattern (Ramos et al., 2013).
Leaf topography is an importance factor for microbial adhesion. The surface roughness of the leaves, crack in the cuticle and other damages are often sites at which bacteria colonize. Leaf stomata provide protective niches for the bacteria. E. coli O157:H7 cells have been found that cells could adhere better to cut lettuce leaf surfaces than intact or normal lettuce leaf surfaces (Seo and Frank, 1999). Many reports showed human pathogen can enter stomata and cut edges of fresh produce (Berger et al., 2010; Golberg et al., 2011)as well as the ability of E. coli O157:H7 and Salmonella to internalize or infect the vascular system of growing plant (Olaimat and Holley, 2012).
Washing fresh produce
Fresh produces washing is a significant step for removing soil, debris, and improving the appearance of the commodity. Washing also to lowering the produces’ temperature to limit the development of physiological changes. Moreover, washing helps to reduce the microbial load on the surface which has impact to quality, shelf-life, and safety of the produces (Herdt and Feng, 2009). Washing produces with water or sanitizing agents could help to remove pesticide residues (Sapers, 2014).
In contrast, washing can transfer microbial contaminants to wash water and also to other uncontaminated raw materials, as well as to the processing equipment (Gil et al., 2009).. Adding sanitizing agents to wash water significantly reduce the population of bacterial cells, and thus the risk of cross-contamination (Sapers, 2014). Washing with sanitizers assist to avoid cross-contamination between clean and contaminated product and significant enhance produce hygiene (Gil et al., 2009). Typically a microbial load of fresh produce can be reduced 1 to 2 logs by washing and disinfection. However, washing is restricted to treat the microorganisms on the surface and internalized microorganisms of fresh produces (Lee et al, 2004).
Not all washing methods and washing solutions are effective (Olaimat and Holley, 2012), the success of washing depends on many factors: type of washing, exposure time, the concentration of sanitizing agent, pH, temperature as well the target microorganisms, the characteristics of produce surface, the attachment of cell to produce surface, the formation of resistant biofilms and the internalization of microorganisms (Allende et al., 2008). The challenges of choosing sanitizing treatment are how to reach pathogens on the surface and in subsurface areas of fresh produce in an active formand and at the same time compromise the quality of fresh produce after treatment (Beuchat, 2004).
Sanitizing agents for fresh produces sanitation
Three factors could influence the efficacy of disinfection need to be noted. Firstly, sanitizing agents including pH, temperature, and water quality; secondly the availability of disinfectant in water which are bound to the inorganic and organic compounds; and lastly the accessibility of sanitizing agents to the target microorganisms (Barbeau et al., 2005).
The chosen of chemical sanitizing agent during the washing disinfection step is a critical step for keeping quality and safety of fresh produces. Ideal sanitizing agent should show sufficient level of antimicrobial activity, and be a negligible effect on the sensory quality of the product (Allende et al., 2008). Most of the common type of sanitizers are oxidizers, working by increasing the oxidation potential in the water system, and are considered to be the Generally Recognized As Safe (GRAS).
Chlorine (Cl) is the most widely sanitizing agent that used to sanitize fresh produce (Sapers, G. M., 2014). It is a very potent disinfectant with powerful oxidizing properties. However an antimicrobial activity of chlorine depends on the pH of wash solution, for the best compromise of activity and stability of hypochlorous acid (HOCl) should be potentially control and maintain at the pH of wash water between 6.5 and 7.5. Currently in the fresh produce industry used Sodium hypochlorite (NaOCl) to disinfectant produce at a concentration of 50-200 mgL-1and a contact time of 1-2 min (Beuchat, 1998;Fan and Sokorai, 2015;Goodburn and Wallace, 2013). Another was reported at 50-150 mg/L (Martínez-Hernández et al., 2015). However the chlorination water containing free chlorine between 20 to 200 mg/L in sanitizing solution cannot eliminate pathogens completely, the reduction of 1 to 3 log CFU/g are common (Aruscavage et al., 2006).
Alternative sanitizing agents used for fresh produce such as electrolyzed oxidizing water, chlorine dioxide, ozone, peroxyacetic acid, acidified sodium chlorite or peracetic acid have been studied (Alvaro et al., 2009;Alwi and Ali, 2014;Artés et al., 2009;Gil et al., 2009;Gómez-lópez, 2012;Goodburn and Wallace, 2013;Graça et al., 2011;Hao et al., 2015; Herdt and Feng, 2009;Olaimat and Holley, 2012;Ramos et al., 2013)
Electrolyzed oxidizing water (EO water or EOW) is an emerging decontamination technology. The EOW system was developed in Japan in 1992 and EOW has been attracted much attention in recent years. It is not only effective solution for inactivation of microorganisms but also is considered as environmentally friendly chemical (Cheng et al., 2012). The role in disinfection mechanism of EOW consists of high oxidation-reduction potential (ORP), pH and available chlorine content (ACC) ae well as the OH radical in EOW (Hao et al., 2015;Hao et al., 2012;Stan and Daeschel, 2005;Stan et al., 2005). The acidic EOW which had a low pH (2.5 to 3.5), high ORP (1000 to 1200 mV), high dissolved oxygen and free chlorine (30 to 90 mg/L) has been regarded as an effectively antimicrobial activity (Ding et al ., 2015; Huang et al., 2008).
Chlorine dioxide (ClO2) is a biocide with 2.5 times the oxidation capacity of chlorine (Rodgers et al., 2004). Chlorine dioxide is highly stable and less corrosive than ozone and chlorine (Joshi et al. , 2013). It can apply as an aqueous solution, or gas phase (Sapers, 2014). Moreover, ClO2 is not active to organic compounds (Han et al., 2004; Lee et al. , 2004). FDA approved the formulation of ClO2 as an antimicrobial agent in water (aqueous ClO2) (21CFR173.300) and can use ClO2 for wash fresh fruit and vegetables. However the residual of ClO2 in final produce should not over than 3 mg/L of ClO2 and suggestion to rinse with potable water after treatment with ClO2 (López-Velasco et al., 2012; Sapers, 2014).
Emerging technology: Fine Bubble Technology
Fine bubble is defined as the small bubbles with a diameter ranging in micro and nanoscale. Microbubbles are the small bubbles with diameter between 10 to 50 µm and decreasing in size and lastly disappear under water (Hideki, 2014; Parmar and Majumder, 2013; Takahashi, 2005; Takahashi et al., 2007), while the nanobubbles is evensmaller bubbles with diameter less than 200 nm (Agarwal et al., 2011). Stabilized nanobubbles are created when microbubbles are collapse in aqueous electrolyte solution (Masayoshi, 2014).
Microbubble composts of three main components, gas phase, shell material, and aqueous or liquid phase. The gas phase is referred to the gas inside the bubbles which may be single gas or the combination of gases. The second is the shell material, an aqueous phase surrounding the gas phase. The formation of the bubbles and the mechanical properties of microbubbles depend on the property of shell material. The last important component is aqueous phases which are the liquid or combined solution surrounding the shell material (Parmar and Majumder, 2013). These three components mainly contribute to the properties of microbubbles.
Several interesting characteristics of microbubbles comparing with a millimeter or centimeter-sized bubbles are described. Although the microbubbles are defined as the bubbles having diameter in order of micrometer (µm) (Hideki, 2014; Parmar and Majumder, 2013), however the range of the bubbles diameter can be varies. Usually the microbubbles have a slow rising speed. Besides, the Reynolds number of microbubbles is nearly 1 and its shape is spherical (Hideki, 2014).
Microbubbles could reduce frictional resistance, therfore the coefficient of friction decreased with increased in volume fraction of microbubbles (Hideki, 2014; Parmar and Majumder, 2013). The internal pressure of bubbles depend on the bubbles diameter and surface tension of bubbles. Decreasing of bubbles diameter caused the increasing of internal pressure inside of the bubbles, that may describe by the Young-Lapace equation. The occurrence of this phenomenon can be explained as increasing the gas dissolution will increase the mass transfer rate due to driving force of gas dissolution in the internal pressure (Parmar and Majumder, 2013). Lastly, decreasing microbubbles in water affect an increasing of the large gas-liquid interfacial area and the changes of the physical properties of water (Hideki, 2014).
Microbubbles have been suggested that they possses a negative charged on their surface (Hideki, 2014; Takahashi, 2005). The studied of the zeta potential of microbubbles in aqueous showed the zeta potential of microbubbles in distilled water was -35 mV (Takahashi, 2005). The zeta potential of the particle is a physical property that is possessed by any particle in suspension and emulsion. Usually the zeta potential of emissions used to predict the stability of suspension and emulsion stability. Likewise, the fine bubbles in the solution similar characteristic like the particle in suspension and having a significant negative potential tend to repel the other particle (Parmar and Majumder, 2013).
Similarly to the nanobubbles, they are tiny bubbles with the diameter in nanoscale and smaller size than microbubbles. It is invisible in solution while microbubbles are visible. Before the generation of microbubbles water is clear and its change from the clear solution to cloudy and milky after the generating the microbubbles (Masayoshi, 2014 ; Li, 2007). Then the smaller bubbles are shrunk faster and disappear almost instantaneously, and the nanobubbles are created after the microbubbles collapse. The half-life of nanobubbles depends on the water condition, many researchers are trying to clarify the mechanism of nanobubbles stability but the difficulty is the measurement the properties of these tiny particles (Tsuge, 2014)
There are many fields application research of microbubbles and nanobubbles such as environmental field, industrial field, agricultural field, medical and food industry due to the different function properties. Recently bubble technology is applied in waste water treatment particularly in food industries, and thus this technology influences the quality, production efficiency or cost of food products. In food process the development of foods using microbubble (MB) or micro-nano bubble (MNB) technology is expected to obtain a new characteristic because the quantity and size of the food bubbles may contribute to their appearance, physical properties and texture of the foods. The application extends to control over the growing and pre-postharvest physiological properties of fresh produce, sterilization of food or equipment, besides the treatment of public water and wastewater (Xu et al., 2014).
Newly developed or quality-improved food/food materials are expected to be produced by microstructure control. Regarding to the process of cream production, Kukizaki (2009) described that controlling the surface characteristics of Fine bubbles (Micro- and nano-Bubbles) will contribute to improving the stability of the emulsion. Apart from that fine bubble is expected to be used as a delivery system of functional or aroma components in foods and used as a carrier of drug or antimicrobial agents to maintain food hygiene or safety. Micro bubbles treatments were applied for sterilization, some biocidal gases are often incorporated, therefore with the combined effect of ozone bubbling and ozone nanobubble treatment have been shown significant microbial efficiency. Moreover the combination microbubbles with ozone gas effectively reduced the pesticide residual on fruits and vegetables ( Ikeura et al, 2011a; 2011b and 2013)
The application Micro/Nano bubble on reducing pesticides in fresh produce
In 2011, Ikeura and coworker compared the efficacy of two types of ozone microbubbles generator, the decompression-type and the gas-water circulating-type, to remove the residual pesticide fenitrothion in lettuce, cherry tomatoes and strawberries. The decompression type produces a sufficient amount of gas that dissolved in water under a 3-4 atmospheric pressure to cause a supersaturated condition. Under such a condition, supersaturated gas is unstable and when escapes from the water generating a lot of air bubbles, which are microbubbles. While the gas–water circulation type, gas is introduced into the water vortex, and the formed gas bubbles are broken into microbubbles by breaking the vortex. They found that the concentration of dissolved ozone was higher in the decompression type solution than in the gas–water circulation type solution, although the concentration of dissolved ozone decreased gradually with time. Washing with ozone microbubbles water effectively removed residual fenitrothion in all vegetable samples (Fig. 1), and the decompression type was shown to be better. Hydroxyl radicals that are generated by the collapse of ozone microbubbles in solutions are highly effective at decomposing organic molecules (Ikura et al., 2011a), resulting in pesticides degradation.
Reduction rate of fenitrothion on cherry tomatoes was slightly different from the other vegetables because the dissolved ozone and hydroxyl radicals could hardly penetrate through the thick pericarp of the cherry tomatoes. While strawberries fruit that have a rougher surface and larger surface area than cherry tomatoes, fruits can contact with ozone efficiently, therefore removing fenitrothion are easily compare to tomatoes. The difference in the pesticide-removing effect between the decompression type and the gas–water circulation type may be caused by the difference in the size and the number of the bubbles (Ikura et al., 2011b).
Later Ikura and co-worker (2011b) found ozone microbubbles (OMCB) produced by continuous microbubble generator could retain the concentration of dissolved ozone at 2.0 ppm (Fig 2.), resulting in effectively removal of fenitrothion better than the ozone millibubble (OMLB). Continuous microbubble generator removes the pesticide better than the bath generation or non-continuous generating. Later different type of pesticides were investigated by Ikeura et al. (2013), fenitrothion is represented as organophosphate insecticide while benomyl is carbamate fungicide. Since allowable pesticides residues in food have been implemented in Japan and other countries, including persimmon wrapping leaves for special sushi in Nara, Japan. Red and green persimmon leaves were chosen as a sample model.
The pesticide-spiked persimmon leaves were subjected to the treatments by different micro bubble generation methods. Ozone concentrations in water varied from 0.2, 0.5, 1.0 and 2 ppm, at immerge times 5, 10 or 15 min, were subjected to leaf samples. The residual of fenitrothion on persimmon leaves were decreased when increased washing time from 5 to 15 min, and again continuous generating the microbubbles was shown better effective removal of the fenitrothion than batch generating, likewise the reduction of benomyl on these leaves. No effect of bubbling or continuous microbubbles during treatment to the color and the pulling strength of Persimmon leaves were found (Ikura et al., 2011b).
Experiment I : Removal of foodborne pathogen in Vegetables using combination of sanitizers and microbubbles. (Mahakarnchanakul et al., 2010)
Six types of popular Thai fresh produce were selected and classified into three types; leafy type: coriander (Coraindrum sativum) and peppermint or Marsh mint or Thai mint (Mentha arvensis); Pod and stem type: asparagus (Asparagus officinalis) and okra (Abelmochus esculentus); Root type: ginger (Zingiber officinale) and lemongrass (Cymbopogon citrates).
All fresh produce were stored at 5°C and placed on the laboratory bench for 30 min, then selected similar size and shape. The 18 h suspension of Salmonella Hvittingfoss and Escherichia coli were prepared for inoculum, the final load on each vegetable type was expected to be 103-104 CFU/ml. No wash before inoculating. Then, vegetables were inoculated by adding culture suspension in Polyethylene bag, manual shaking for 2 min, placing under a laminate flow cabinet to let them dry for 30 min. Samples were analyzed for initial loads.
Micro bubble water was prepared by circulating the tap water through a generator in sterile plastic tank (5L). MB generator was obtained from Shigen Kaihatsu Co., Ltd., Japan. Five hundred grams of each sample was submerged in 5 L of micro bubble water (at flow rate of 4.5 L/min) by ratio 1:10 and, agitating for 15 min. Then vegetables were drained and dried under laminate flow cabinet at 20± 3oC (65-70% relative humidity) for 30 min before microbial analyze.
Total viable counts were determined on Plate Count Agar (PCA) while E. coli was counted on McConkey agar, likewise S. Hvittingfoss on Xylose lysine desoxycholate (XLD). Plates were incubated at 35 °C for 24 h before enumeration the survivors (Figure 4). All samples were enumerated by rinse test to avoid the natural antimicrobial from smashed vegetables.
The fragile leaves of coriander and Marsh mint are easily damaged from the harsh washing process. Washing by using MB water showed distinctively good appearance and fresh green leaves due to dissolved oxygen during bubbles generating. The reduction of microbial population by washing with micro bubble water was better than normal washing with tap water. MB treatments could reduce microflora on coriander by 87.6% log reduction, while tap water could reduce microflora load about 56% log reduction. While washing Marsh mint showed microbial reduction by tap water and micro bubbles treatment as 95 and 80 % reduction, respectively (Figure 3A and 3B).
Thai asparagus and okra were known as major exporting produce particularly for Japan market. Washing with MB water and tap water could reduce natural microflora on asparagus and okra. The reductions of total microbial on asparagus and okra using MB water were 99.6 and 98 % compared to washing with tap water, which gave reduction less by 92 and 95 %. (Figure 3C, and 3D). The MB showed the high efficiency in reducing normal flora in asparagus and okra by 1.7-2.4 log CFU/g.
In case of root type, lemongrass and ginger, normally the natural load was high as 6-7 log CFU/ml due to the nature of root plants. Rough and cracked surfaces of ginger prone to be heavy contaminated with soil and difficult to remove soil, resulting in high microbial population and E. coli presence. Fig 3E and 3F showed the remarkably reduction of microflora on lemongrass and ginger samples. Before washing with MB, microflora on lemongrass and ginger were 5.8 and 6.8 log CFU/ml and after washing population was reduced to 4.3 and 5.8 log CFU/ml (97 and 89% reduction). Washing lemongrass and ginger using tap water showed slightly less reduction by 93 to 94%.
In conclusion the number of total bacterial count (microflora) deposited on surface of tested vegetables was contaminated from 105 to 107 CFU/ml, microbial population was varies depending on the different physiology of plant. Each type of vegetables has different in texture and level of microbial contamination, besides the presence of small fur on Marsh mint leaves, or the crevices of surfaces of ginger resulting in the possible habitat. The chance of soil contact during harvesting may also cause the high microbial contamination in produce. The efficiency of MB washing varied from 78 to 99.6% and presented better microbial reduction comparing to tap water wash at 56 to 95.6%, respectively.
Artificially inoculation acquired the initial load of Escherichia coli (E. coli) and Salmonella on coriander and Marsh mint by 2.5 to 3.2 and 4.2 to 4.6 log CFU/ml. The reductions of E. coli and Salmonella on coriander after MB washing are shown in Figure 4A and 4B. Populations of E. coli on coriander decreased more than 90.0% or 1 log reduction after washing with MNB water, as well as tap water. Salmonella on coriander washed with MNB water and tap water was decreased by 99.5% and 99.0%, respectively.
The effect of MB water and tap water for reducing Marsh mint contaminated with E. coli and Salmonella were shown in Figure 4C and 4D. E. coli was reduced by 95.3 % reductions after MB washing, while tap water was by 73%. Results showed better Salmonella reduction by 98 to 99.8 % with MB water and tap water. Different level reduction between these two strains may result from their ability to attach on produce surface which may affect to less washing out, particularly E. coli.
The best reduction among the six types of tested vegetables was found in asparagus inoculated with E. coli. Completely reduction from the initial of 2.2-2.7 resulting in 100 % reduction (not detect at dilution of 10-1) after washing with MB and tap water (Fig 5A). However the effect of washing might not be great compared to Salmonella. Washing with MB water and tap water assisted to reduce Salmonella populations on asparagus by 74 and 80 % (Figure 5B). Likewise less reduction when washing okra with MB and tap water, microbial reduction was 52 to 86%. Great result was shown when using MB as washing water, 100% Salmonella reduction on okra while using tap water gave 98 %. (Fig 5C and 5D)
The initial population of E. coli and Salmonella on Lemongrass were 2.4 to 2.5 log CFU/ml and 3.3 to 3.7 log CFU/ml (Fig 6A and 6B). Reduction of Salmonella was 98 % reduction. MB water washing could be the promising method to reduce E. coli and Salmonella on lemongrass, as well as 98 % reduction on E. coli and 90% Salmonella on ginger (Fig 6C and 6D).
The advantage of using MNB water as washing agent is the possible on less chemical agent added. This preliminary study was conducted to investigate the possibility and feasibility of Micro bubble water washing to replace chemical in order to sanitize fresh produce. The results revealed that MB water contribute to the promising washing agents on microbial reduction which may be potentially applied to the industry and food services.
However, it was found that additional factors such as water flow rate and washing time assisted to reduce the microbial load. Application in industry need to consider all factors involved in washing produce. Apart from the chemical use, the success of the washing depends on several factors such as target microorganisms, characteristics of produce surface, attachment of cells to produce surface, formation of resistant biofilms and internalization of microorganisms (Allende et al., 2008).
Expeiment II: Effect of combined microbubbles and various sanitizer agents to reduce Escherichia coli on Chinese kale (Klintham et al., 2013)
E. coli TISTR780 and Chinese kale was chosen as the model in this study. The final load on Chinese kale was 5-6 log CFU/mg. The ratio of 200g of Chinese kale per 400 ml of culture suspension was mixed in PE plastic bag and then let it soaked for 15 min, before let them dry in laminar flow cabinet for 20 min. Chinese kale samples were MB treated and the samples were taken for E. coli analysis. Washing condition was done as; 100g of Chinese kale to wash water 10000 mL, with washing time 5 min under continuous generated the microbubbles.
The combination treatments between generated microbubbles and various concentration of NaOCl (50 ppm and 100 ppm), Acetic acid and Citric acid (0.5% and 1.0% w/v) in washing contaminated kale showed remarkably the efficiency of sanitizer to eliminate E. coli on Chinese kale compared to normal washing by tap water. Using the microbubbles water without or with chemical added have no significantly affect microbial reduction, especially E. coli from Chinese kale, however, increasing the concentration of sanitizers (NaOCl, Acetic acid and Citric acid) in washing process contributed to be better microbial reduction (Fig. 7).
Recent experiment: Determination on the efficacy of fine bubbles combined with sanitizers on reducing Salmonella Typhimurium and Escherichia coli on Thai Mint and Sweet Basil
Recent study by Klintham et al. 2014 (unpublished) was performed to determine the effect of washing by using the fine bubbles combined with two types of sanitizers on reducing Salmonella Typhimurium and Escherichia coli on Thai Mint and Sweet Basil. Acidic electrolyte water (20 and 40 ppm free chlorine, AEO), and chlorine dioxide (3 and 5 ppm ClO2) combined with batch bubble generated by IDEC (Model UFBGALF™) were investigated. The condition of washing was at 5 min with shaking (60 rpm at 25±2°C).
Determination of oxidation-reduction potential (ORP) in AEO at 20 and 40 ppm AEO were 1090-1200 mV (pH 2.9-3.0) and 1110-1260 mV (pH 2.7-2.9), respectively, which presented active oxidation, while chlorine dioxide has less ORP value, as 750-850 mV at 3 ppm (pH 5.8), and 810-890 mV at 5 ppm (pH 5.1). After washing ORP value were decreased although the pH of wash water were slightly increased. Using 40 ppm AEO with fine bubble treatment effectively reduced Salmonellae cells on sweet basil and Thai mint with 90.7% and 99.5% log reduction respectively (P≤0.05). Compared to the results on microbial reduction by aqueous ClO2, when combined with fine bubbles, washing treatments could reduce Salmonellae on sweet basil and Thai mint with 99.0% and 90.3% log reduction respectively. Noticeably, E. coli washing with AEO was reduced to less than 74 % and 86 %, similar to the effect of 5 ppm ClO2 on E. coli reduction (9% and 16%). None of microbes was observed in wash water, which showed that adding these two sanitizers in bubble water resulting in strong antimicrobial effects on planktonic bacteria. Although tap water with and without FB also showed microbial reduction, but there are still viable cells 5-6 log10 CFU/ml found in wash water .
Conclusions
Fine bubble technology provides the opportunities to apply in agriculture and food products. Using of the fine bubbles technology has unclear mechanism on pesticides degradation, but Japanese laboratory showed remarkably pesticides reduction when combining fine bubbles with oxidizing agents like ozone. Recently Thai research group applied combination of fine bubbles with chemicals like chlorine compounds, organic acids, and others oxidizing agents. Results showed this technology assist to reduce the pathogenic microorganisms in fresh vegetables. More studies need to be done to further clarify the antimicrobial mechanism, and to control possible factors to maximize the utilization of this technology. This technology has the potential to decrease chemical use, and may be considered as the friendly environmental technology. Lastly, the challenge of the fine bubbles technology is to find appropriate use, and to apply to other food process.
References
Agarwal, A., W.J. Ng and Y. Liu, 2011. Principle and applications of microbubble and nanobubble technology for water treatment. Chemosphere 84(9): 1175-1180.
Allende, A., M.V. Selma, F. López-Gálvez, R. Villaescusa and M.I. Gil, 2008. Role of commercial sanitizers and washing systems on epiphytic microorganisms and sensory quality of fresh-cut escarole and lettuce. Postharvest Biology and Technology 49(1): 155-163.
Alvaro, J.E., S. Moreno, F. Dianez, M. Santos, G. Carrasco and M. Urrestarazu, 2009. Effects of peracetic Acid disinfectant on the postharvest of some fresh vegetables. Journal of Food Engineering 95(1): 11-15.
Alwi, N.A. and A. Ali, 2014. Reduction of Escherichia coli O157, Listeria monocytogenes and Salmonella enterica Sv. Typhimurium populations on fresh-cut bell pepper using gaseous ozone. Food Control 46(0): 304-311.
Aruscavage, D., K. Lee, S. Miller and J.T. LeJeune, 2006. Interactions affecting the proliferation and control of human pathogens on edible plants. Journal of Food Science. 71(8): R89-R99.
Barbeau, B.,R. Desjardins, C. Mysore and M. Prévost, 2005. Impacts of water quality on chlorine and chlorine Dioxide efficacy in natural waters. Water Research 39(10): 2024-2033.
Berger, C.N., S.V. Sodha, R.K. Shaw, P.M. Griffin, D. Pink, P. Hand and G. Frankel, 2010. Fresh fruit and vegetables as vehicles for the transmission of human pathogens. Environ Microbiol 12(9): 2385-2397.
Beuchat, L.R.,1998. Surface decontamination of fruits and vegetables eaten raw: A review. Food safety unit, World Health Organization.
Beuchat, L.R. 2002, Ecological factors influencing survival and growth of human pathogens on raw fruits and vegetables. Microbes and Infection 4(4): 413-423.
Cheng, K.C., S.R.S. Dev, K.L. Bialka and A. Demirci, 2012. Chapter 19-Electrolyzed oxidizing water for microbial decontamination of food pp. 563-591, in A. Demirci and M. O. Ngadi, eds. Microbial Decontamination in the Food Industry. Woodhead Publishing.
Delbeke, S., S. Ceuppens, L. Jacxsens and M. Uyttendaele, 2015. Microbiological analysis of pre-packed sweet basil (Ocimum basilicum) and Coriander (Coriandrum sativum) leaves for the presence of Salmonella spp. and shiga toxin-producing E. coli. International Journal of Food Microbiology 208(0): 11-18.
Ding, T., Z. Ge, J. Shi, Y.-T. Xu, C.L. Jones and D.-H. Liu, 2015. Impact of slightly acidic electrolyzed water (Saew) and ultrasound on microbial loads and quality of fresh fruits. LWT - Food Science and Technology 60(2, Part 2): 1195-1199.
Elviss, N.C., C.L. Little, L. Hucklesby, S. Sagoo, S. Surman-Lee, E. de Pinna and E.J. Threlfall, 2009. Microbiological study of fresh herbs from retail premises uncovers an international outbreak of Salmonellosis. International Journal of Food Microbiology 134(1-2): 83-88.
Fan, X. and K. J. Sokorai, 2015. Formation of trichloromethane in chlorinated water and fresh-cut produce and as a result of reaction with citric acid. Postharvest Biology and Technology 109(0): 65-72.
Gil, M.I., M.V. Selma, F. Lopez-Galvez and A. Allende, 2009. Fresh-cut product sanitation and wash water disinfection: Problems and solutions. Int J Food Microbiol 134(1-2): 37-45.
Golberg, D., Y. Kroupitski, E. Belausov, R. Pinto and S. Sela, 2011. Salmonella Typhimurium internalization is variable in leafy vegetables and fresh herbs. Int J Food Microbiol 145(1): 250-257.
Gómez-López, V.M., M.I. Gil, L. Pupunat and A. Allende, 2015. Cross-contamination of Escherichia coli O157:H7 is inhibited by electrolyzed water combined with salt under dynamic conditions of increasing organic matter. Food Microbiology 46(0): 471-478.
Goodburn, C. and C.A. Wallace, 2013. The microbiological efficacy of decontamination methodologies for fresh produce: A review. Food Control 32(2): 418-427.
Graça, A., M. Abadias, M. Salazar and C. Nunes, 2011. The use of electrolyzed water as a disinfectant for minimally processed apples. Postharvest Biology and Technology 61(2-3): 172-177.
Han, Y., T.L. Selby, K.K. Schultze, P.E. Nelson and R.H. Linton, 2004. Decontamination of strawberries using batch and continuous chlorine dioxide gas treatments. Journal of Food Protection 67(11): 2450-2455.
Hao, J., S. Qiu, H. Li, T. Chen, H. Liu and L. Li, 2012. Roles of hydroxyl radicals in electrolyzed oxidizing water (EOW) for the inactivation of Escherichia coli. International Journal of Food Microbiology 155(3): 99-104.
Hideki, T., 2014. Characteristics of microbubbles pp. 3-10, in. H. Tsuge (ed.) Micro- and Nanobubbles:Fundamental and application. Pan Stanford Publishing Pte. Ltd.
Huang, Y.-R., Y.-C. Hung, S.-Y. Hsu, Y.-W. Huang and D.-F. Hwang, 2008. Application of electrolyzed water in the food Industry. Food Control 19(4): 329-345.
Ikeura, H., F. Kobayashi and M. Tamaki, 2011a. Removal of residual pesticides in vegetables using ozone microbubbles. J Hazard Matter 186(1): 956-959.
Ikeura, H., F. Kobayashi and M. Tamaki, 2011b. Removal of residual pesticide, Fenitrothion, in vegetables by using ozone microbubbles generated by different methods. Journal of Food Engineering 103(3): 345-349.
Ikeura, H., S. Hamasaki and M. Tamaki, 2013. Effects of ozone microbubble treatment on removal of residual pesticides and quality of Persimmon leaves. Food Chem 138(1): 366-371.
Joshi, K., R. Mahendran, K. Alagusundaram, T. Norton and B.K. Tiwari, 2013. Novel disinfectants for fresh produce. Trends in Food Science & Technology 34(1): 54-61.
Herdt, J. and H. Feng, 2009. Aqueous antimicrobial treatments to improve fresh and fresh - cut produce safety pp. 433 in Xuetong F., B. A. Niemira, C. J. Doona, F. E. Feeherry and R. B. Gravani (eds). Microbial Safety of Fresh Produce. Blackwell Publishing and the Institute of Food Technologists. USA.
Kirezieva, K., P.A. Luning, L. Jacxsens, A. Allende, G.S. Johannessen, E.C. Tondo, A. Rajkovic, M. Uyttendaele and M.A.J.S. van Boekel, 2015. Factors affecting the status of food safety management systems in the global fresh produce chain. Food Control 52(0): 85-97.
Lee, S.-Y., M. Costello and D.-H. Kang, 2004. Efficacy of chlorine dioxide gas as a sanitizer of lettuce leaves. Journal of Food Protection 67(7): 1371-1376.
López-Velasco, G., A. Tomás-Callejas, A. Sbodio, F. Artés-Hernández and T.V. Suslow, 2012. Chlorine dioxide dose, water quality and temperature affect the oxidative status of tomato processing water and its ability to inactivate Salmonella. Food Control 26(1): 28-35.
Martínez-Hernández, G.B., J. Navarro-Rico, P.A. Gómez, M. Otón, F. Artés and F. Artés-Hernández, 2015. Combined sustainable sanitising treatments to reduce Escherichia coli and Salmonella Enteritidis growth on fresh-cut Kailan-hybrid Broccoli. Food Control 47(0): 312-317.
Mahakarnchanakul, W., P. Klintham1, S. Tongchitpakdee and W. Chinsirikul, 2010. Micro-/Nano- Bubble Technology: An Alternative for sanitizing fresh fruits and vegetables and other potential applications in Thailand. Chapter 1. Feasibility Study on Using Micro-/Nano- Bubble Water to Eliminate Foodborne Pathogen on Fresh Produce. Research project under National Metal and Materials Technology Center (MTEC) National Science and Technology Development Agency (NSTDA) and NEDO. NEDO report. Thailand.
Klintham, P., W. Mahakarnchanakul, S. Tongchitpakdee and W. Chinsirikul, 2013. Application of microbubbles in washing process fro microbial reduction on Thai fresh vegetables pp. 56. in Proceeding of the International Conference on Postharvest Technology, Food chemistry and Processing.: Developing the Supply Chain towards More Healthy Food. 11-13 Nov 2013, at Hanoi University of Agriculture, Hanoi, Vietnam.
Masayoshi, T. 2014. Nanobubbles: An Introduction pp. 307-315, in. H. Tsuge (ed.) Micro- and Nanobubbles:Fundamental and application. Pan Stanford Publishing Pte. Ltd.
Olaimat, A.N. and R.A. Holley, 2012. Factors influencing the microbial safety of fresh produce: A review. Food Microbiol 32(1): 1-19.
Parmar, R. and S.K. Majumder, 2013. Microbubble generation and microbubble-aided transport process intensification-a state-of-the-art report. Chemical Engineering and Processing: Process Intensification 64(79-97).
Ramos, B., F.A. Miller, T.R.S. Brandão, P. Teixeira and C.L.M. Silva, 2013. Fresh fruits and vegetables-an overview on applied methodologies to improve its quality and safety. Innovative Food Science & Emerging Technologies 20(0): 1-15.
Rodgers, S.L., J.N. Cash, M. Siddiq and E.T. Ryser, 2004. A comparison of different chemical sanitizers for inactivating Escherichia coli O157:H7 and Listeria monocytogenes in solution and on apples, lettuce, strawberries, and cantaloupe. Journal of Food Protection 67(4): 721-731.
Sapers, G.M., 2014. Chapter 17 - Disinfection of contaminated produce with conventional washing and sanitizing technology pp. 389-431, in K. R. Matthews, G. M. Sapers and C. P. Gerba (eds). The Produce Contamination Problem. Academic Press, San Diego, USA
Seo, K.H. and J.F. Frank, 1999. Attachment of Escherichia coli O157:H7 to lettuce leaf surface and bacterial viability in response to chlorine treatment as demonstrated by using confocal scanning laser microscopy. Journal of Food Protection 62(1): 3-9.
Takahashi, M., 2005. Ζ Potential of microbubbles in aqueous solutions: Electrical properties of the gas−water interface. The Journal of Physical Chemistry B. 109(46): 21858-21864.
Takahashi, M., K.Chiba and P. Li, 2007. Free-radical generation from collapsing microbubbles in the absence of a dynamic stimulus. The Journal of Physical Chemistry B. 111(6): 1343-1347.
Takahashi, M.,2014. Chapter 11 Nanobubbles:An introduction pp.307-317, in. H. Tsuge (ed.) Micro- and Nanobubbles:Fundamental and application. Pan Stanford Publishing Pte. Ltd.
Tsuge, H.,2014. Chapter 2 Formation and analysis ofmicrobubbles pp.11-83, in. H. Tsuge (ed.) Micro- and Nanobubbles:Fundamental and application. Pan Stanford Publishing Pte. Ltd.
Xu, Q., N. Nobutaka and T. Shiina, 2014. Chapter 7 Food Industry pp.207-217, in. H. Tsuge (ed.) Micro- and Nanobubbles:Fundamental and application. Pan Stanford Publishing Pte. Ltd.
Xuetong, F., B.A.N., C.J. Doona, F.E. Feeherry and R. B. Gravani, 2009. Microbial Safety of Fresh Produce, pp. 445. in J. H. Feng, eds. Aqueous Antimicrobial Treatment to Improve Fresh and Fresh-Cut Produce Safety. Blackwell Publishing and Institute of Food Technologist. USA.
Fig. 1. The residual percentage of fenitrothion (FT) in lettuce (A), cherry tomatoes (B) and strawberries (C) at 5 and 10 min after the immersion into the solutions of ozone micro bubble (OMB) treatments by using the gas–water circulation type and the decompression type. Modified from: Ikeura et al., 2011a.
Fig. 2. The residual fenithothion (FT) on lettuce, cherry tomatoes and strawberry treated with the Ozone microbubbles (OMCB) and Ozone millibubbles (OMLB) solutions. Modified from: Ikeura et al., 2011b.
Fig. 3. Populations of total microbial count on un-inoculum coriander (3A), Marsh mint (3B), asparagus (3C), okra (3D), lemongrass (3E), and ginger (3F) before (0 min) and after (15 min) washing by tap water and MNB water.
Ref: Mahakarnchanakul et al., 2010.
Fig. 4. Populations of E. coli on coriander (4A), Marsh mint (4C), and population of Salmonella on coriander (4B), Marsh mint (4D) before (0 min) and after (15 min) washing by tap water and MNB water.
Ref: Mahakarnchanakul et al., 2010.
Fig. 5. Populations of E. coli on asparagus (5A), okra (5C), and population of Salmonella on asparagus (5B), okra (5D) before (0 min) and after (15 min) washing by tap water and MNB water.
Ref: Mahakarnchanakul et al., 2010
Fig. 6. Populations of E. coli on asparagus (6A), okra (6C), and population of Salmonella on asparagus (6B), okra (6D) before (0 min) and after (15 min) washing by tap water and MNB water.
Ref: Mahakarnchanakul et al., 2010.
Fig. 7. Survivor of E.coli on Chinese kale after treatments.
Ref: Klintham et al., 2013.